Because of its component parts, a description of the biomechanics of the shoulder complex is rather involved. To make the subject at once comprehensive and relevant to the clinician, the structure and function of the sternoclavicular and acromioclavicular joints are dealt with first. The anatomy and biomechanics of the glenohumeral joint are then discussed in three parts according to an outline familiar to clinicians: motion, constraints, and forces across the joint (Table 6-1). By way of classification, we have adopted the increasingly accepted phrase of arm elevation rather than abduction or flexion, when possible. Although this organization is somewhat arbitrary and has some overlap, it does allow a reasonably simple and logical approach to understanding the entire shoulder complex.
Clinical Function | Biomechanical Description |
Motion | Kinematics |
Stability | Constraints |
Strength | Force transmission |
SHOULDER COMPLEX
The function of the shoulder girdle requires the integrated motion of the sternoclavicular, acromioclavicular, glenohumeral, and scapulothoracic joints. This motion is created by the delicate interaction of almost 30 muscles that control the total system complex. Discussion of the biomechanics of this complex focuses first on the sternoclavicular and acromioclavicular joints and then on the glenohumeral and scapulothoracic joints.
Sternoclavicular Joint
According to Dempster, six actions occur at the sternoclavicular joint: elevation, depression, protrusion, retraction, and upward and downward rotation.1 The amount of potential motion present at this articulation has been studied by disarticulating the scapula. Anteroposterior rotation exceeds superoinferior motion by about 2 : 1.2 In an intact and functioning extremity, the actual amount of displacement is of course limited by the attachment to the scapula; this motion is described later. At the extremes, motion is limited by tension developed in the ligamentous complex on the opposite side of the joint. This constraint occurs in concert with increased contact pressure at the articulation and the intra-articular disk ligament (Fig. 6-1). Approximately 35 degrees of upward rotation occurs at the sternoclavicular joint.3,4 A similar 35 degrees of anterior and posterior rotation and up to 45 to 50 degrees of axial rotation also occur at this joint.
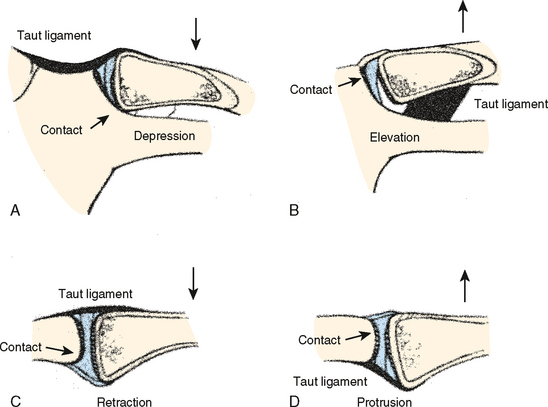
FIGURE 6-1 Superior (A), inferior (B), anterior (C), and posterior (D) ligaments stabilize the sternoclavicular joint by enhancing the contact force at certain joint positions.
(Redrawn from Dempster WT: Mechanisms of shoulder movement. Arch Phys Med Rehabil 46A:49-70, 1965.)
Anterior displacement of the distal end of the clavicle is not affected by release of the interclavicular or costoclavicular ligaments or by the intra-articular disk.5 Release of the capsular ligament is followed by downward displacement of the lateral aspect of the clavicle.
Inferior displacement of the clavicle at the sternum is resisted by articular contact at the inferior aspect of the joint and by tautness developed in the interclavicular ligament and the posterior expansion of the capsular ligament.1 Superior translation of the joint is resisted by tension developed in the entire costoclavicular complex. Little, if any, articular constraint assists in resisting this displacement.
Protrusion or anterior displacement of the clavicle is resisted not only by the anterior capsule but also by the posterior portion of the interclavicular ligament and the posterior sternoclavicular ligament. Conversely, posterior displacement or retraction of the medial aspect of the clavicle generates tension in the anterior portion of the inferior capsular ligaments and in the anterior sternoclavicular ligament, as well as in the anterior portion of the costoclavicular ligament. Only a limited amount of articular contact resists such displacement; this contact is on the posterior vertical ridge of the sternal articulation and the posterior portion of the clavicle. Spencer and colleagues demonstrated that the posterior capsule was the most important stabilizer for both the anterior and posterior translations of the medial end of the clavicle, whereas the anterior capsule was also important for anterior translation.6 According to them, the costoclavicular and interclavicular ligaments had little effect on the anterior-to-posterior stability of this joint.
Clavicular rotation causes twisting of the capsular ligaments. Only about 10 degrees of downward (forward) rotation occurs before the ligaments become taut and limit further motion. With upward (backward) rotation of the clavicle, up to 45 degrees of rotation occurs before the entire complex again becomes taut and resists further rotatory displacement.7 Both these actions increase compression across the sternoclavicular joint. The costoclavicular ligament is thought to be the most important single constraint in limiting motion at this joint.8
Acromioclavicular Joint
Motion and Constraint
The amount of possible acromioclavicular motion that is independent of the sternoclavicular link has been found to be limited by the complex arrangement of the coracoclavicular and acromioclavicular ligaments. According to most investigators, rotation of the acromioclavicular joint takes place about three axes.1,3,8,9 These motions are variously described but can simply be termed anteroposterior rotation of the clavicle on the scapula, superoinferior rotation, and anterior (inferior) and posterior (superior) axial rotation. Of these three, anteroposterior rotation of the clavicle with respect to the acromion is approximately three times as great as superoinferior rotation of an intact specimen.10 Sahara and colleagues quantified anterior-to-posterior and superior-to-inferior translations of the distal end of the clavicle on the acromion using open MRI. They reported that the distal end of the clavicle translated most posteriorly (average 1.9 mm) at 90 degrees of abduction and most anteriorly (average 1.6 mm) at maximum abduction. The superior-to-inferior translation was much smaller, with the distal end of the clavicle shifted slightly superiorly (average 0.9 mm) during arm elevation.
Regarding the constraints of the acromioclavicular joint, Dempster noted that the conoid and trapezoid became taut with anteroposterior scapular rotation, thus serving as the constraint of this motion.1 In a subsequent study by Fukuda and coworkers, however, the acromioclavicular ligament was noted to be taut in its anterior component with posterior rotation and taught in its posterior component with anterior rotation.9 Anterior rotation of the clavicle with regard to the scapula was also found to cause some tightness in the conoid and trapezoid ligaments, but this tightness was of a magnitude approximately equal to the stretch observed in the posterior acromioclavicular ligament. Thus, the limiting factor to this motion is the posterior component of the acromioclavicular ligament. On the other hand, posterior rotation of the clavicle is restrained only by the anterior fibers of the acromioclavicular ligament, with virtually no contribution from the other structures.
Superoinferior rotation of the clavicle is quite limited at the acromioclavicular joint. Both Dempster and Kapandji noted virtually no ligamentous contribution to resisting inferior displacement (Fig. 6-2).1,8 However, superior rotation of the clavicle with respect to the acromion is limited primarily by the medial aspect of the conoid ligament, with subsequent tightening of the lateral portion of this structure (Fig. 6-3). The trapezoid provides approximately the same degree of constraint as do the anterior and posterior portions of the acromioclavicular ligament complex. Once again, the magnitude of this displacement is not reported by either investigator but is said to be limited.
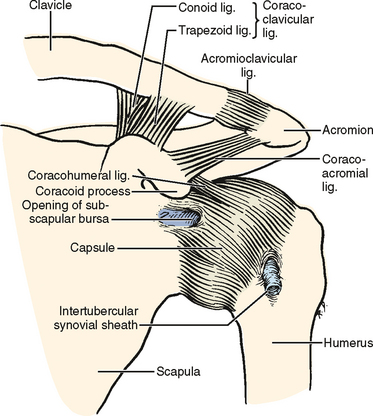
FIGURE 6-3 The coracoclavicular ligament complex consists of the larger and heavier trapezoid ligament, which is oriented laterally, and the smaller conoid ligament, which is situated more medially.
(Modified from Hollinshead WH: Anatomy for Surgeons, vol 3. New York: Harper & Row, 1969.)
Anterior and posterior axial rotation (inferior to superior) was reported by Dempster to be about 60 degrees in cadaveric shoulders without the thorax.1 In live shoulders, Rockwood and Green demonstrated only 5 to 8 degrees of motion at the acromioclavicular joint using two Kirschner wires inserted into the acromion and the clavicle.7According to the recent three-dimensional kinematic analysis using open MRI, Sahara and colleagues demonstrated in volunteer shoulders that the anterior axial rotation of the clavicle at the acromioclavicular joint increased linearly with abduction and reached 35 degrees on average at maximum abduction of the arm.11
Both anterior and posterior axial rotations are limited by the conoid ligament. Posterior axial rotation is accompanied by tightening of the trapezoid ligament, with some contribution from the medial and anterior conoid as well as from the acromioclavicular ligament complex.9 Dempster, however, indicates that the acromioclavicular ligament is taut in the extreme of anterior and posterior axial rotation and is a limiting factor of this motion.1 Fukuda and colleagues have quantified the displacement as a function of the ligamentous constraints.9 Slight displacement is limited by the acromioclavicular ligament, but large displacements are resisted by the coracoclavicular ligaments (Fig. 6-4).
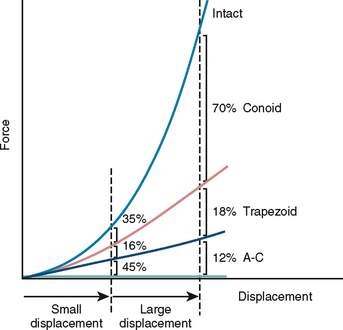
FIGURE 6-4 All displacements are resisted by the force generated in the acromioclavicular ligament, and large displacements are also resisted by the conoid and trapezoid structures. A-C, acromioclavicular.
(Redrawn from Fukuda K, Craig EV, An K-N, et al: Biomechanical study of the ligamentous system of the acromioclavicular joint. J Bone Joint Surg Am 68:434-440, 1986.)
Relative contributions of the individual capsular ligaments (anterior, posterior, superior, and inferior) were studied by Klimkiewicz and colleagues, who demonstrated that the superior and posterior acromioclavicular ligaments were the most important contributors to the acromioclavicular restraint against posterior translation of the distal end of the clavicle (56% and 25%, respectively).12
Debski and coworkers also investigated the capsular contribution to stability of the acromioclavicular joint. When the capsule was released, significant anterior-to-posterior instability was observed but not superior-to-inferior instability.13 Capsular release increased loading on the coracoclavicular ligaments: Anterior load increased tension in the conoid ligament, and posterior load increased tension in the trapezoid ligament. Thus, the trapezoid and conoid ligaments have different functions.
Motion of the Clavicle
The potential motion present at the sternoclavicular and acromioclavicular joints exceeds that actually attained during active motion of the shoulder complex. Current data indicate that accurate demonstration of the phasic three-dimensional motion of the sternoclavicular and acromioclavicular joints that occurs with arm elevation is a complex problem.14 During elevation of the extremity, clavicular elevation of about 30 degrees occurs, with the maximum at about 130 degrees of elevation (Fig. 6-5).3 The clavicle also rotates forward approximately 10 degrees during the first 40 degrees of elevation. No change takes place during the next 90 degrees of elevation, but an additional 15 to 20 degrees of forward rotation subsequently occurs during the terminal arc. Forward elevation (flexion) demonstrates virtually an identical pattern of clavicular motion.
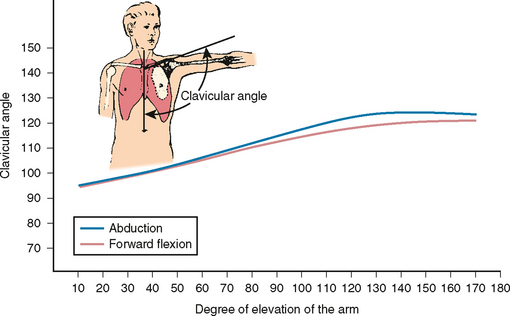
(Redrawn from Inman VT, Saunders JR, Abbott LC: Observations on the function of the shoulder joint. J Bone Joint Surg 26:1-30, 1944.)
Axial rotation of the clavicle is reported by Inman and coworkers to be an essential and fundamental feature of shoulder motion, particularly arm elevation (Fig. 6-6). If the clavicle is not allowed to rotate, elevation of only about 110 degrees is said to be possible.3 Superior (posterior) rotation of the clavicle begins after the arm has attained an arc of about 90 degrees of elevation and then progresses in a rather linear fashion, with approximately 40 degrees of rotation attained at full elevation (see Fig. 6-6).3 These findings have been challenged by Rockwood and Green. Placement of pins in the clavicle and acromion shows less than 10 degrees of rotation with full arm elevation.7 This discrepancy suggests that more than 30 degrees of axial rotation occurs at the sternoclavicular joint.
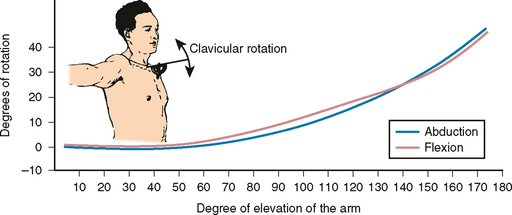
(Redrawn from Inman VT, Saunders JR, Abbott LC: Observations on the function of the shoulder joint. J Bone Joint Surg 26:1-30, 1944.)
Sahara and coworkers, in contrast, reported that 35 degrees of axial rotation occurred at the acromioclavicular joint, indicating that less rotation occurred at the sternoclavicular joint.11 Clinically, fixation of the clavicle to the coracoid by a screw does not greatly limit shoulder elevation and ankylosis caused by ectopic bone also causes minimal loss of arm elevation (Fig. 6-7). The patient shown in Figure 6-7 could elevate his arm to about 160 degrees. On the other hand, ankylosis of the sternoclavicular joint allows only 90 degrees of shoulder elevation.7 Thus, loss of motion at the acromioclavicular joint appears to be better tolerated than loss of motion at the sternoclavicular joint.
Clinical Relevance
Acromioclavicular instability is one of the most important and controversial topics clinically relevant to the shoulder. The acromioclavicular capsular ligament complex is the primary constraint for small rotational displacements at this joint. Downward force applied to the end of the scapula causes inferior displacement of the acromion (grade III injury) and thus violates the constraint provided by the conoid and trapezoid ligaments. Lesser degrees of ligamentous disruption, such as occur with grade I or II acromioclavicular sprains, demonstrate minimal or no inferior migration of the acromion. Biomechanical data have explained this finding by showing that the conoid ligament must be intact to prevent even slight displacement.
Resection of the distal clavicle is commonly performed in patients with osteoarthritis of the acromioclavicular joint. Debski and colleagues reported that acromioplasty did not affect kinematics of this joint, but acromioplasty together with distal clavicle resection increased posterior translation by 30% during posterior loading and increased the in situ force in the trapezoid and conoid ligaments almost three times greater than in the intact shoulder during anterior loading.15 Corteen and Teitge also reported that resection of the distal clavicle increased posterior translation by 32%.16 Reconstruction using the coracoacromial ligament significantly stabilized the acromioclavicular joint. Thus, the significant effect of distal clavicle resection on motion and ligament forces should be taken into consideration when this procedure is to be used.
GLENOHUMERAL AND SCAPULOTHORACIC JOINT MOTION
The motion of the shoulder complex is probably greater than that of any other joint in the body. The arm can move through an angle of approximately 0 to 180 degrees in elevation, internal and external rotation of approximately 150 degrees is possible, and flexion and extension—or anterior and posterior rotation in the horizontal plane—is approximately 170 degrees.17 This motion, which represents the composite motion of several joints, occurs primarily in the glenohumeral and scapulothoracic joints; extreme positions require rotation at the sternoclavicular and acromioclavicular joints.
Motion of the shoulder complex has been a topic of concern and controversy for more than 100 years. Reasons for this debate are numerous and include imperfect devices or means of measurement because the soft tissue envelope makes it difficult to actually observe the skeletal motion, confusion with respect to terminology, inconsistency in defining the reference system, and an early lack of understanding of the concept of sequence-dependent serial rotation. Early investigations focused on arm motion about the sagittal, coronal, and transverse planes. However, because the sequence-dependent nature of rotation about orthogonal axes was not appreciated, years of debate and discussion centered on understanding and explaining Codman’s paradox.
Codman’s Paradox
Codman’s paradox may be demonstrated easily (Fig. 6-8). From the resting position in the anatomic posture with the medial epicondyle pointing toward the midline of the body, the arm is brought forward to 90 degrees of flexion and abducted 90 degrees. The epicondyle is now pointing perpendicular to the coronal plane. The arm is then brought back to the side to its apparent initial position, but the medial epicondyle is now observed to be rotated anteriorly away from the body instead of medially toward the midline of the body. The humerus, however, was never axially rotated.18
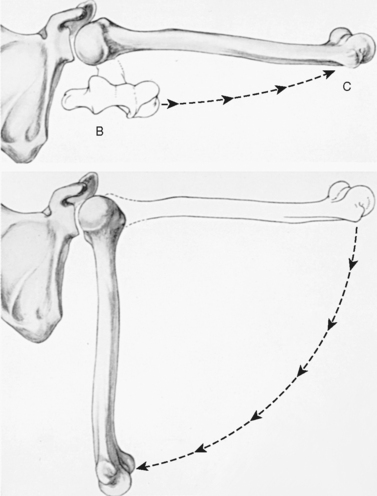
FIGURE 6-8 Codman’s paradox. Top, The humerus is flexed to a right angle (B) and swung backward to the plane of the scapula (C). Bottom, It is then brought back to the vertical. An axial rotation position change has occurred without actual axial rotation taking place. (From Johnston TB: The movements of the shoulder joint. A plea for the use of the “plane of the scapula” as the plane of reference for movements occurring at the humero-scapular joint. Br J Surg 25:252-260, 1937.)
The difficulty in understanding this phenomenon has prompted numerous discussions.18–21 The simplest explanation is that serial angular rotations are not additive but are sequence dependent, which means that 90 degrees of rotation about the z-axis and then the x-axis results in a different final position than does rotation about the x-axis and then the z-axis (Fig. 6-9). Multiple rotations about orthogonal axes must therefore be defined by the sequence of the rotation. In aerospace terms, these rotations are called the Eulerian angles: yaw, pitch, and roll.
Techniques to Observe and Describe Motion of the Shoulder Complex
Because of the complexity of this issue and the conflicting results, it is appropriate to describe various techniques that have been used to measure upper extremity motion. Methods of observing and describing motion of the upper extremities may be broadly categorized into research and clinical effort.
The early research effort to describe shoulder joint (complex) motion consisted of simple (but careful) observation of cadaveric material and thus often included observation of the ligamentous constraints.18,22–24 A gross description of motion and displacement has proved to be accurate even today because of the careful nature of these early observations. With the advent of the roentgenogram, uniplanar25 and biplanar cineradiographic studies are the more common techniques used today for active and passive investigations. These techniques are particularly attractive because they can be used in vivo. By implanting metal markers, very accurate three-dimensional rotation may be measured from these radiographs.26 With the advent of computer data manipulation, replication of motion by using a complex system involving an interactive microcomputer for analyzing images with real-time graphic display has been developed. This method and other modeling techniques are much too complex for routine use but can serve as valuable research tools.27,28
Clinical measurement techniques include simple and complex goniometers. Doody and associates designed a goniometer to be used in vivo that measures glenohumeral and scapulothoracic motion simultaneously.24,29 Electrogoniometers have not been of routine clinical value but have been used extensively for basic science investigations. Unfortunately, the anatomy constraints at the shoulder limit the value of electrogoniometers. A stereometric method has also been used for three-dimensional kinematic analysis. Basically, when three non-colinear points fixed to a rigid body are defined within an inertial reference frame, the position and orientation of that rigid body can be specified and the relative rotation and translation occurring at a joint can be determined. Numerous commercial systems using light-emitting diodes, reflecting dots, and ultrasonic transducer techniques are available for such an application.
Aerospace technology has provided a device that uses three mutually orthogonal magnetic fields30; it has proved useful as both a research and a clinical tool. This instrument has been applied to in vivo and in vitro studies and measures simultaneous three-dimensional rotational motion.31,32 In addition, translation displacement has also been calculated, thus allowing determination of the screw axis, which defines the complete displacement characteristics of the system.
More recently, Sahara and colleagues used magnetic resonance images to measure three-dimensional kinematics of the glenohumeral joint.33 Magnetic resonance images were obtained with volunteers in a seated position and in seven static positions of the arm from 0 degrees to maximum abduction using vertically open magnetic resonance imaging. Three-dimensional surface models were created and three-dimensional movements of each bone in the glenohumeral joint were calculated using a computer algorithm. This is a noninvasive method, and in vivo kinematics of the bony structures can be precisely measured.
Description of Joint Motion
The aforementioned techniques permit joint motion to be described with varying degrees of sophistication. In general, joint kinematics may be divided into two-dimensional planar motion and three-dimensional spatial motion. With planar motion, the moving segment both translates and rotates around the fixed segment. A more distinctive description of planar motion, however, can be based on rotation around a point or axis, which is defined as the instantaneous center of rotation (ICR). Theoretically, the ICR could be determined accurately if the velocities of points on the rigid body are measurable. In practice, an alternative technique based on the method of Rouleaux is commonly adopted. In this method, the instantaneous locations of two points on the moving segment are identified from two consecutive positions within a short period of time, and the intersection of the bisectors of the lines joining the same points at the two positions defines the ICR (Fig. 6-10).
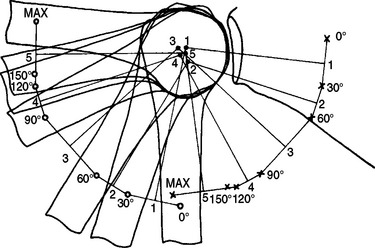
FIGURE 6-10 Measurement of the center of rotation of the humeral head as defined by the Rouleaux technique. MAX, maximum.
(From Walker PS: Human Joints and Their Artificial Replacements. Springfield, Ill: Charles C Thomas, 1977.)
Occasionally it is useful to describe the planar joint articulating motion.34 For general planar or gliding motion of the articular surface, the terms sliding, spinning, and rolling are commonly used (Fig. 6-11).
Sliding motion is defined as pure translation of a moving segment against the surface of a fixed segment. The contact point of the moving segment does not change, but its mating surface has a constantly changing contact point. If the surface of the fixed segment is flat, the ICR is located at infinity; otherwise, it is at the center of the curvature of the fixed surface.
Spinning motion is the exact opposite of sliding motion; the moving segment rotates and the contact point on the fixed surface does not change. The ICR, in this case, is located at the center of curvature of the spinning body that is undergoing pure rotation.
Rolling motion is motion between moving and fixed segments in which the contact points on each surface are constantly changing. However, the arc length of the moving surface matches the path on the fixed surface so that the two surfaces have point-to-point contact without slippage. The relative motion of rolling is a combination of translation and rotation. The ICR is located at the contact point.
Three-Dimensional Glenohumeral Joint Motion
Three-dimensional analysis of motion of a rigid body requires three linear and three angular coordinates to specify the location and orientation of a rigid body in space. In other words, any rigid body with unconstrained motion has six degrees of freedom in space. Numerous methods are available to describe spatial rigid body motion, two of the most commonly used of which are description of the Eulerian angle and the screw displacement axis.
If the glenohumeral joint is stable and the motion can be assumed to be that of a ball-and-socket joint, it is sufficient to consider only rotation of the joint and neglect small amounts of translation. In this case, description of three-dimensional rotation by using the Eulerian angle system is most appropriate (Fig. 6-12). It should be remembered, as emphasized earlier, that general three-dimensional rotation is sequence dependent. In other words, with the same specified amount of rotation around three axes, the final result will be different if the sequence of the axes of rotation is different, which is one of the explanations for Codman’s paradox.
With the arm hanging at the side of the body, the z-axis is defined to be perpendicular to the scapular plane. The y-axis points out laterally and the x-axis points distally along the humeral shaft axis. The rotational sequence for the Eulerian description of glenohumeral joint rotation or the orientation of the humerus relative to the scapula is as follows: First rotate the humerus around the x-axis by an amount f to define the plane of elevation. Then rotate the arm around the rotated z (z′)-axis by an amount q to define the arm elevation. Finally, axial rotation of the humerus around the rotated x (x″)-axis by an amount ψ completes the process.
During circumduction motion of the humerus, for example, the corresponding Eulerian angle could be measured as shown in Figure 6-12. This description could be used clinically to describe the range of joint motion as well as the specification of joint position at which any abnormality or pathologic process should be documented.
In instances in which a more general description of glenohumeral joint displacement is required, the screw displacement axis (SDA) description is most appropriate. The rotation and translation components of displacement of the humerus relative to the glenoid or scapula are defined by rotation around and translation along a unique screw axis(Fig. 6-13). In addition to incorporating a description of translation, the advantage of using the SDA method is that the orientation of the SDA remains invariant regardless of the reference coordinate axes used. The SDA can be determined experimentally with various methods. With a rotational matrix describing the orientation and a positional vector from a reference point known for the rigid body, the SDA can be calculated.35 If the coordinates of at least three reference points on the rigid body are measured, the SDA can also be calculated.36
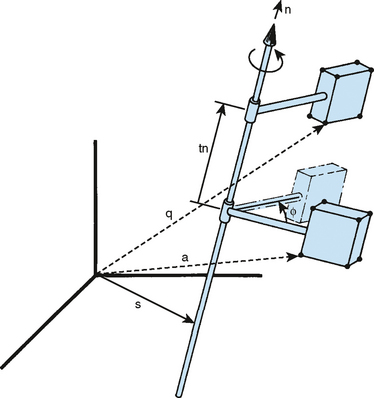
FIGURE 6-13 Both rotational (Φ) and translational (tn) components of displacement of a rigid body may be expressed by the concept of the screw axis (n), which represents the shortest path from position a to position q around or along which the displacement can be described. s, shortest distance from the center of coordinate system to the screw axis.
SHOULDER MOTION
Resting Posture
Scapula
The resting position of the scapula relative to the trunk is anteriorly rotated about 30 degrees with respect to the frontal plane as viewed from above (Fig. 6-14). The scapula is also rotated upward about 3 degrees with respect to the sagittal plane as viewed from the back (Fig. 6-15).17,37 Finally, it is tilted forward (anteflexed) about 20 degrees with respect to the frontal plane when viewed from the side.14 This posture of the scapula is not influenced by an external load (up to 20 kg) applied to the extremity.14
McClure and colleagues measured the three-dimensional kinematics of the scapula during dynamic movement of the shoulder. A three-dimensional motion sensor was firmly fixed to the scapula with a Kirschner wire.38 During arm elevation in the scapular plane, the scapula upwardly rotated (average of 50 degrees), tilted posteriorly around a medial-lateral axis (30 degrees), and externally rotated around a vertical axis (24 degrees). Lowering of the arm resulted in reversal of these motions in a slightly different pattern. The mean ratio of glenohumeral to scapulothoracic motion was 1.7:1. The researchers concluded that normal scapular motion consists of substantial rotation around three axes, not simply upward rotation. Using the same method, Bourne and colleagues reported similar results.39
Fung and associates measured scapular and clavicular kinematics during passive humeral motion.40 Scapular and clavicular rotation was relatively small until the humerus reached approximately 90 degrees of elevation. The glenohumeral-to-scapulothoracic ratio was approximately 2 for the entire range of elevation for each elevation plane, but it was dramatically larger during early elevation than during late elevation.
Humerus
The humeral head rests in the center of the glenoid when viewed in the plane of the glenoid surface.18,41 Fick referred to this relationship as Nullmeridianebene, or dead meridian plane.41 The humeral head and shaft are thought to lie in the plane of the scapula. The 30-degree retroversion of the articular orientation is complemented by the 30-degree anterior rotation of the scapula on the trunk.
Articular Surface and Orientation
Humerus
The articular surface of the humerus constitutes approximately one third the surface of a sphere with an arc of about 120 degrees. This articular surface is oriented with an upward tilt of approximately 45 degrees and is retroverted approximately 30 degrees with respect to the condylar line of the distal end of the humerus (Fig. 6-16).1,17,41,42Retroversion of the humerus is much greater in children.43 The average retroversion is 65 degrees between 4 months and 4 years of age and 38 degrees between 10 and 12 years of age. Most of the derotation process takes place by the age of 8 years, with the remainder developing gradually until adulthood. This derotation process seems to be restricted in young throwing athletes,44 resulting in increased retroversion in dominant arms.45,46
Glenoid
In the coronal plane, the articular surface of the glenoid comprises an arc of approximately 75 degrees. The shape of the articulation is that of an inverted comma. The typical long-axis dimension is about 3.5 to 4 cm. In the transverse plane, the arc of curvature of the glenoid is only about 50 degrees, with a linear dimension of approximately 2.5 to 3 cm.41 The relationship of the articular surface to the body of the scapula is difficult to define precisely because of the difficulty in defining a frame of reference. Typically, it is accepted that the glenoid has a slight upward tilt of about 5 degrees47 with respect to the medial border of the scapula and is retroverted a mean of approximately 7 degrees, although individual variation in these measurements is considerable (Fig. 6-17).48
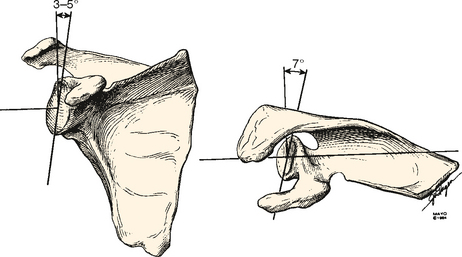
FIGURE 6-17 The glenoid faces slightly superior and posterior (retroverted) with respect to the body of the scapula.
(Modified from Mayo Clinic © 1984.)
Saha has defined the relationship of the dimensions of the humeral head and the glenoid as the glenohumeral ratio. This relationship is approximately 0.8 in the coronal plane and 0.6 in the horizontal or transverse plane.48 These values are consistent with several observations that have estimated that only about one third of the surface of the humeral head is in contact with the glenoid at any given time.17 Hertz49 measured the surface area of the glenoid with and without the labrum and compared it with that of the humeral head. The surface ratio of the glenoid and humeral head was 1:4.3 without the labrum and 1:2.8 with the labrum. In other words, the glenoid surface with the labrum attached is approximately one third the humeral head surface, and it is approximately one fourth the humeral head surface without the labrum.
Arm Elevation
The most important function of the shoulder—arm elevation—has been extensively studied to determine the relationship and contribution of the glenohu-meral and scapulothoracic joints, the scapulohumeral rhythm.3,24,25,42,48,50–55 Although early descriptions of scapulohumeral rhythm were based on motion with respect to the coronal (frontal) plane, recent discussion has defined this motion with respect to the scapular plane. Neither reference system is completely adequate to fully describe the complex rotational sequences involved in elevation of the arm because the changes in scapular position during elevation usually have not been considered.
Early descriptions of this motion defined the glenohumeral contribution as the first 90 degrees, followed by scapulothoracic rotation.17 Subsequent discussions place the overall glenohumeral-to-scapulothoracic motion ratio at 2:1.3,14 This ratio is inconsistent during the first 30 degrees of elevation, with variation by person and even by sex.21,29,52
Poppen and Walker reported a 4:1 glenohumeral-to-scapulothoracic motion ratio during the first 25 degrees of arm elevation.25 Thereafter, an almost equal 5:4 rotation ratio occurs during subsequent elevation. The average overall ratio is about 2:1.
The lack of linearity of this motion complex has also been observed by Doody and coworkers, who showed a 7:1 ratio of scapulothoracic-to-glenohumeral motion during the first 30 degrees of elevation and an approximately 1:1 ratio from 90 to 150 degrees of arm elevation.29 Others have also shown nonlinear variation during elevation.55Further evaluation of the arm against resistance elicits scapulothoracic motion earlier than with passive motion alone.29
The various studies have been simply summarized by Bergmann.56 During the first 30 degrees of elevation, variably greater motion occurs at the glenohumeral joint. The last 60 degrees occurs with about an equal contribution of glenohumeral and scapulothoracic motion. The overall ratio throughout the entire arc of elevation is about 2:1 (Fig. 6-18).
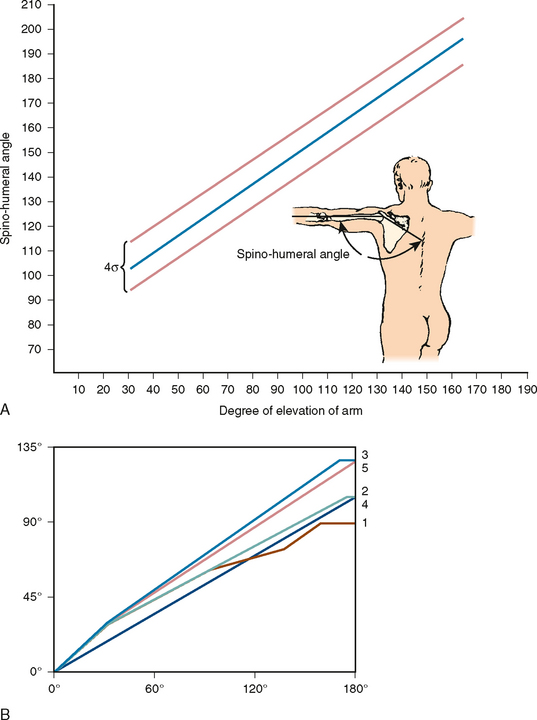
FIGURE 6-18 A, The classic study by Inman and colleagues shows the relationship between glenohumeral and scapulothoracic motion. The blue line indicates the regression line. The red lines indicate the range of ±2 SD. B, Angular changes of the glenohumeral joint with respect to arm elevation were determined by several investigators. 1, Nobuhara K: The Shoulder: Its Function and Clinical Aspects. Tokyo: Igaku-Shoin, 1987. 2, Poppen NK, Walker PS: Normal and abnormal motion of the shoulder. J Bone Joint Surg Am 58:195-201, 1976. 3, Inman VT, Saunders JR, Abbott LC: Observations on the function of the shoulder joint. J Bone Joint Surg 26:1-30, 1944. 4, Freedman L, Munro RH: Abduction of the arm in scapular plane: Scapular and glenohumeral movements. J Bone Joint Surg Am 18:1503-1510, 1966. 5, Reeves B, Jobbins B, Flowers M: Biomechanical problems in the development of a total shoulder endoprosthesis. (Proc Br Orthop Res Soc) J Bone Joint Surg [Br] 54:193, 1972. (A, Redrawn from Inman VT, Saunders JR, Abbott LC: Observations on the function of the shoulder joint. J Bone Joint Surg 26:1-30, 1944. B, Redrawn from Bergmann G: Biomechanics and pathomechanics of the shoulder joint with reference to prosthetic joint replacement. In Koelbel R, Helbig B, Blauth W [eds]: Shoulder Replacement. Berlin: Springer-Verlag, 1987, pp 33-43.)
Harryman and associates confirmed this ratio for planes other than the scapular or coronal plane.57 After measuring the three-dimensional kinematics of the glenohumeral and scapulothoracic joints in various planes of elevation, they concluded that the relative contribution of glenohumeral and scapulothoracic motion to the total arc of elevation was consistent and essentially 2:1. The scapulohumeral rhythm is affected by the speed of arm elevation.58 At high speed, glenohumeral motion is more dominant at the beginning of motion. The rhythm remains the same, although the total range of motion is reduced with age.59
With upward movement of the arm, a complex rotational motion of the scapula occurs (Fig. 6-19). In addition to the upward rotation described earlier, about 6 degrees of anterior rotation with respect to the thorax occurs during the first 90 degrees of arm elevation. Posterior rotation of about 16 degrees occurs next, with the scapula coming to rest about 10 degrees posteriorly rotated in comparison to the original resting position.50 Thus, an arc of about 15 degrees of anteroposterior rotation of the scapula occurs with elevation of the arm; about 20 degrees of forward tilt with respect to the thorax also occurs during elevation.14 Scapulohumeral rhythm is affected by various pathologic conditions of the shoulder. In stiff shoulders with anterior capsular tightness, a more excessive scapular upward rotation is observed.60 On the other hand, shoulders with instability show delay in retraction and posterior tilt of the scapula during arm elevation, which can contribute to shoulder instability.61 Scapulohumeral rhythm changes after total shoulder arthroplasty, with the 2:1 ratio changed to 1:2 after nonconstrained total shoulder arthroplasty.62
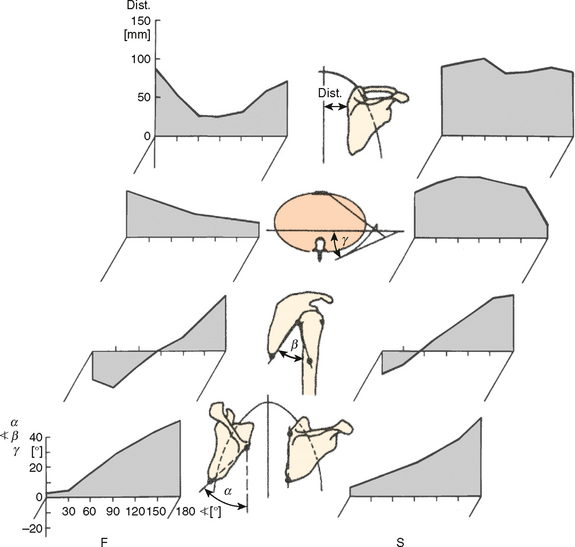
FIGURE 6-19 Complex three-dimensional rotation and translation of the scapula during arm elevation from 0 to 180 degrees in the frontal plane (F) and sagittal plane (S). α, scapular rotation in the frontal plane; β, scapular rotation in the sagittal plane; γ, scapular rotation in the horizontal plane; Dist, distance between the vertical at C7 and the medial border of the scapular spine.
(Modified from Laumann U: Kinesiology of the shoulder joint. In Koelbel R, Helbig B, Blauth W [eds]: Shoulder Replacement. Berlin: Springer-Verlag, 1987, pp 23-31.)
External Rotation of the Humerus
Early observers noted that “obligatory” external rotation of the humerus was necessary for maximal elevation.18 Impingement of the tuberosity on the coracoacromial arch was assumed to be the mechanical constraint. External rotation clears the tuberosity posteriorly, thereby allowing full arm elevation (Fig. 6-20).18 We have observed in our laboratory that external rotation of the humerus also loosens the inferior ligaments of the glenohumeral joint. This mechanism thus releases the inferior checkrein effect and allows full elevation of the arm. Full elevation with maximal external rotation has also been shown to be a position of greater stability of the shoulder than the elevated position.51
Browne and associates63 quantified the relationship between elevation and rotation of the humerus with respect to the fixed scapula by using a three-dimensional magnetic tracking device. The plane of maximal arm elevation was shown to occur 23 degrees anterior to the plane of the scapula. Elevation in any plane anterior to the scapular plane required external rotation of the humerus, and maximal elevation was associated with approximately 35 degrees of external rotation. Conversely, maximal glenohumeral elevation with the arm in full internal rotation occurs in a plane about 20 to 30 degrees posterior to that of the scapula and is limited to only about 115 degrees.63 They reported that the observed effects of this rotation were to clear the humeral tuberosity from abutting beneath the acromion and to relax the inferior capsuloligamentous constraints. Gagey and Boisrenoult also reported that the inferior capsule determines the maximum abduction angle.64 On the other hand, Jobe and Iannotti reported that obligatory external rotation occurs not because of abutment between the greater tuberosity and the acromion but because of abutment between the greater tuberosity and the superior posterior glenoid rim.65 This concept was first described by Walch and colleagues as posterosuperior impingement66 and was later known as internal impingement.
In vivo measurements of maximal elevation by Pearl and colleagues67 revealed a slight difference from these cadaver studies. According to them, maximal elevation was achieved with the humerus just behind the scapular plane (−4 degrees). A difference in definition of the scapular plane and a difference between in vivo behavior and that of the cadaver might explain the discrepancy.
The complex sequence of events in combined motion of the glenohumeral and scapulothoracic joints has been divided into four stages. Glenohumeral motion occurs first; next, sternoclavicular and then acromioclavicular rotation is observed with elevation of the scapula; and finally, the scapula pivots upward around the acromioclavicular joint. This simplified analytic description is, in general, consistent with the observations of Laumann, Nobuhara, and others.14,55
Center of Rotation
An accurate calculation of the ICR of the humeral head is a complex problem that is much simplified if the motion is limited to a single plane.68,69 Such has been the assumption of most analyses. Hence, the center of rotation of the glenohumeral joint has been defined as a locus of points situated within 6 ± 2 mm of the geometric center of the humeral head (see Fig. 6-10).25 This definition, generated by the Rouleaux technique, is considered reasonably accurate.70 However, this particular technique for defining the center of rotation is accurate only for pure spinning motion, is subject to input-type error,71 and is not accurate in pathologic conditions in which translation is a significant component of the displacement or in which a significant amount of nonplanar motion is present. These limitations might explain why other authors have found the center to lie 8 mm behind and 6 mm below the intersection of the shaft and head axes.72 Still others have reported that multiple centers of rotation occur during abduction.73
The relatively small dimension of this locus as well as the relative consistency of its definition as lying in the geometric center of the humeral head reflects the small amount of translation that normally occurs at this joint and is consistent with the aforementioned observations. A small amount (∼3 mm) of upward translation has been reported in the intact shoulder during the first 30 degrees of elevation; only about 1 mm of additional excursion occurs with elevation measured at greater than 30 degrees.25 A small amount of translation has also been confirmed in cadaver models. During passive elevation without force to the muscles, the humeral head shifted superiorly by 0.35 to 1.2 mm.31,74 By using simulated muscle force to the deltoid and rotator cuff muscles, greater superior-to-inferior translation of the humeral head was recorded (2.0-9.0 mm).75,76Furthermore, an increase in translation occurs with certain pathologic processes such as rotator cuff deficiency25 and tendon rupture of the long head of the biceps (LHB).77
The center of rotation of the scapula for arm elevation is situated at the tip of the acromion as viewed edge on (Fig. 6-21).78
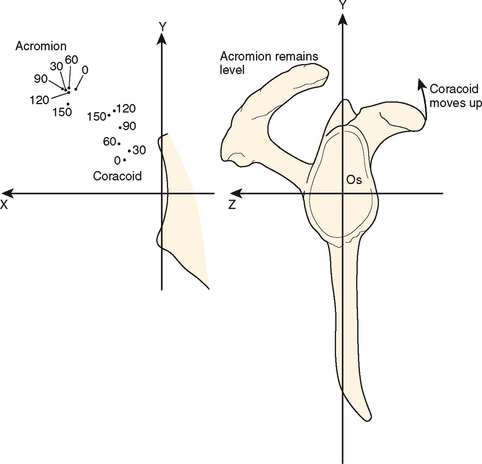
FIGURE 6-21 The center of rotation of the scapula for arm elevation is focused in the tip of the acromion. Left, Anterposterior view. Right, Lateral view. Os, reference point of the scapula (center of the glenoid). (Redrawn from Poppen NK, Walker PS: Normal and abnormal motion of the shoulder. J Bone Joint Surg Am 58:195-201, 1976.)
Screw Axis
Application of the SDA for glenohumeral joint motion has one specific advantage. By using the concept of the intersection or the middle point of the common perpendicular between two instantaneous screw axes as the measurement of the three-dimensional ICR, the stability or laxity of the joint can be described. If the joint is tight and stable, the points of intersection of all the screw axes will be confined within a small sphere (Fig. 6-22). On the other hand, when the joint is becoming unstable because of disease of either the capsuloligamentous structures or the rotator cuff, the points of intersection of the screw axes will be more dispersed and confined in a larger sphere. Stokdijk and colleagues compared different methods in determining the glenohumeral joint rotation center in vivo, and they prefer the screw axes method as a reliable and valid method in movement registration.79 The concept had also been used to measure the anterior instability of the shoulder at the end of the late preparatory phase of throwing.80
Clinical Relevance
Understanding of the biomechanical features discussed earlier has several clinically relevant applications. The orientation of the scapula and humerus with respect to the thorax and to each other has been important in designing the optimal radiographic studies to best visualize the scapulohumeral relationship. Thus, the true anteroposterior radiograph of the glenohumeral joint is taken 30 degrees oblique to the sagittal plane. Some think that this orientation is closer to 45 degrees because this angle produces a better true anteroposterior radiographic study.7 The scapular view is taken at a 30-degree angle to the frontal plane; thus, the anteroposterior radiograph (Fig. 6-23) that is perpendicular to this view is taken at an angle of about 60 degrees to the thorax.81
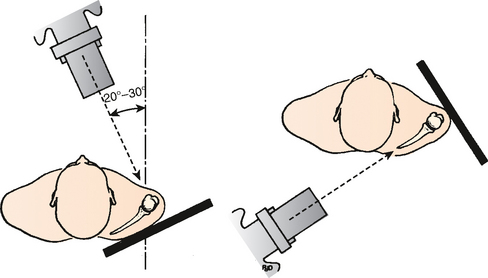
FIGURE 6-23 The anterior (left) and lateral (right) views of the glenohumeral joint were defined by Neer based on knowledge of the scapulothoracic orientation.
(Modified from Neer CS II: Displaced proximal humeral fractures. I. Classification and evaluation. J Bone Joint Surg Am 52:1077-1089, 1970.)
The relationship of coupled external rotation with maximal arm elevation helps explain, to some extent, the limitation in elevation that is seen with a frozen shoulder. To the extent that this condition results in limitation of external rotation, an even more severe restriction of arm elevation is likely to occur. In fact, any simple movement of the glenohumeral joint results in coupled motion in two additional planes. By using a universal full-circle goniometer to determine the relationship between flexion and rotation at the glenohumeral joint it was found that flexion was accomplished by internal rotation.82 It has also been demonstrated that certain passive motions of the glenohumeral joint were reproducibly accompanied by translation of the humeral head on the glenoid.31 Knowledge of this coupling effect is also important with respect to prescribing the appropriate physical therapy after certain surgical procedures or pathologic states.
Arthrodesis of the shoulder is an effective procedure but is most efficacious if the fusion is performed in the appropriate position.83 Although the optimal position is debated, the basis of the selection depends on normal scapulothoracic motion (Fig. 6-24). This knowledge, combined with an understanding of the motion required for activities of daily living, dictates the position of the fusion.
The potential for scapulothoracic motion provides an explanation for the remaining motion of the shoulder girdle present with a frozen shoulder and after arthrodesis. In addition, rotation of the scapula may be viewed as a means of providing a glenohumeral relationship that allows the deltoid muscle to remain effective even with the arm fully elevated (Fig. 6-25).
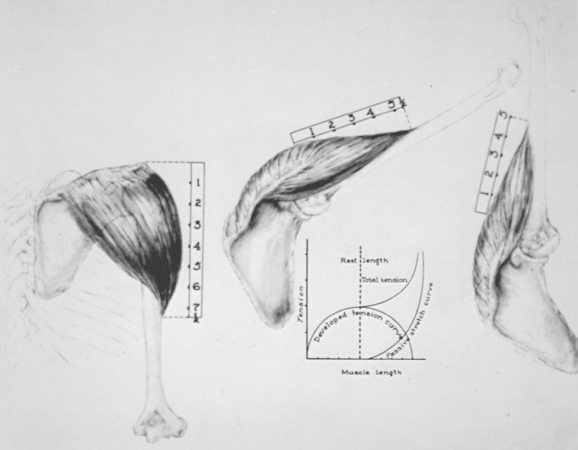
FIGURE 6-25 Scapulothoracic motion allows the deltoid to remain in the optimal position for effective contraction throughout the arc of arm elevation.
(From Lucas DB: Biomechanics of the shoulder joint. Arch Surg 107:425-432, 1973. © 1973, American Medical Association.)
Understanding the axis of rotation is important for prosthetic replacement of the glenohumeral joint. The relative lack of translation in an intact shoulder justifies the design of an unconstrained glenoid surface. Karduna and associates84 reported a significant increase in superoinferior and anteroposterior translation with increased radial mismatch of the prosthesis. The mean translation for natural joints was best reproduced by implant joints with a 3- to 4-mm radial mismatch. To the extent that the cuff musculature is deficient, a greater amount of translation is anticipated, which places additional requirements on the optimal glenoid design to accommodate the increased translation.85 As is discussed later, probably more important is that the initiation of shoulder abduction results in forces directed toward the superior rim of the glenoid; this has implications regarding the stability, force, and optimal prosthetic design and surgical implantation technique.
Finally, the well-recognized superior translation of the humeral head in a rotator cuff–deficient shoulder is explained in part by the superiorly directed resultant vector that occurs with initiation of abduction by the intact deltoid, the lack of soft tissue interposition of the rotator cuff (Fig. 6-26),86 and the lack of centralizing force to the humeral head against the glenoid socket produced by the rotator cuff. Yamaguchi and colleagues measured the kinematics of the glenohumeral joint with symptomatic and asymptomatic rotator cuff tears.87 They found similar superior migration of the humeral head during arm elevation in both symptomatic and asymptomatic rotator cuff tears. Symptoms in shoulders with a rotator cuff tear may be related to factors other than superior migration of the humeral head. In addition to the size of the tear and the duration of symptoms, the important factor that influences superior migration of the humeral head is fatty degeneration of the infraspinatus muscle.88
SHOULDER CONSTRAINTS
It is convenient to consider the constraints of any joint as consisting of static and dynamic elements. The static contribution may be further subdivided into articular and capsuloligamentous components (Box 6-1). Knowledge of the shoulder constraints is of particular clinical interest because it pertains to anterior dislocation as well as to posterior and multidirectional instability of the shoulder.23,89
Early investigators focused on one element or the other. Hence, Saha emphasized the articular component of shoulder stability,48,90 Moseley and Overgaard91 and Townley70 focused on the capsuloligamentous complex, and DePalma and others emphasized the dynamic contribution of the interrelationship between the dynamic and the static capsuloligamentous constraints.92–94 Since the 1990s, the static and dynamic components have been extensively investigated. In addition, the interrelationship between these components has become clarified in both experimental and clinical settings.
In the mid range of motion, the capsuloligamentous structures are lax and the humeral head is movable anteriorly, posteriorly, or inferiorly by applying force. This mobility is called laxity. There is a great variety in the midrange laxity: Some patients are very stiff, but others can dislocate their shoulders voluntarily without any symptoms. The midrange stabilizers are the intra-articular pressure when all the muscles are relaxed; when the muscles are in contraction, the midrange stabilizer is the concavity of the glenoid. On the other hand, translation of the humeral head at the end range of motion is quite limited because the capsuloligamentous structures are tight and prevent further movement of the arm. At this limit of motion, an excessive force can cause failure of the capsuloligamentous structures, which results in traumatic dislocation of the shoulder.
The end-range stabilizers are the capsuloligamentous structures. The midrange stabilizers and the end-range stabilizers are independent. For example, a shoulder with hyperlaxity can reveal an inferior dislocation of the shoulder with the arm in hanging position (midrange laxity), but it might not be dislocated with the arm in an apprehension position of abduction and maximum external rotation (end-range stability). Conversely, a shoulder with recurrent anterior dislocation does not show inferior subluxation or dislocation with the arm in hanging position because the end-range stabilizers are not intact but the midrange stabilizers are intact.
Static Constraints
Articular Contribution to Glenohumeral Stability
The humeral articular surface is not inherently stable. The 30-degree retroversion is obviously necessary for proper balance of the soft tissues and normal kinematics. Most studies of the articular contribution to shoulder stability have focused on the glenoid. The glenoid articulation demonstrates a slight, but definite posterior or retroverted orientation averaging about 7 degrees with regard to the body of the scapula (see Fig. 6-17). Saha has emphasized that this orientation is an important contribution to stability of the joint.48 Recent biomechanical studies have revealed that an anteverted glenoid component results in increased anterior translation of the humeral head and a retroverted glenoid component increases posterior translation of the humeral head,95 whereas compensatory anteversion of the humeral component does not increase shoulder stability.96Theoretically, version of the glenoid could be a predisposing factor for instability. In the clinical setting, however, anterior shoulder instability often observed as a result of traumatic dislocation is not associated with anteversion of the glenoid,97 whereas posterior shoulder instability often observed as a symptom of atraumatic multidirectional instability is associated with increased retroversion of the glenoid.98
Only 25% to 30% of the humeral head is covered by the glenoid surface in any given anatomic position.17,42,49,92 The dimensional relationship between the humeral head and the glenoid reflects the inherent instability of the joint and has been referred to as the glenohumeral index, which is calculated as the maximal diameter of the glenoid divided by the maximal diameter of the humeral head. Saha reported this ratio to be approximately 0.75 in the sagittal plane and approximately 0.6 in the more critical transverse plane.48 Later, this relationship was redetermined, with similar values of 0.86 and 0.58, respectively.99 Developmental hypoplasia of the glenoid can alter this ratio and might play some role in recurrent dislocation of the shoulder, but such observations have been rather limited in the clinical literature.100,101 Subtle variation in articular anatomy of the glenoid has also been described and has been advocated as an explanation for inherent instability of the joint (Fig. 6-27).
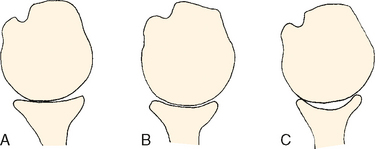
FIGURE 6-27 Articular stability of the glenohumeral joint is enhanced or lessened according to the variation in articular congruence. A, Shallow glenoid surface. B, Conforming surfaces. C, Excessively deepened glenoid surface.
(Modified from Saha AK: Dynamic stability of the glenohumeral joint. Acta Orthop Scand 42:491-505, 1971.)
The glenoid labrum has three layers of collagen fibers.102 The thin superficial layer (articular side) is composed of reticulated collagen fibers, the second layer is composed of stratified collagen fibers, and the third is composed of dense collagen fibers running parallel to each other and oblique to the glenoid rim. The glenoid labrum increases the area and depth of the glenoid cavity. The area of the glenoid with the labrum attached is approximately one third the humeral articular surface, and it is one quarter without the labrum.49 The area of the labrum decreases with age, but the area of the osseous glenoid does not change (Fig. 6-28). The depth of the glenoid is also functionally deepened by the presence of the glenoid labrum.
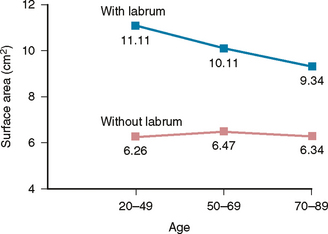
(Redrawn from Hertz H: Hertz H: Die Bedeutung des Limbus glenoidalis für die Stabilität des Schultergelenks. Wien Klin Wochenschr Suppl 152:1-23, 1984.)
The early literature placed little emphasis on this anatomic structure as increasing the stability offered by the glenoid articular surface. Townley70 removed the labrum of cadaveric shoulders through a posterior approach but could not create anterior dislocation until he resected the anterior capsule. Moseley and Overgaard demonstrated that the labrum was a specialized portion of the anterior capsule.91 With external rotation, this structure flattens and thus serves only as a source of attachment for the inferior glenohumeral ligament (IGHL); hence, they concluded that the labrum itself seems to offer little to the inherent stability of the joint. However, other studies have attributed additional importance to the labrum.94 Howell and coworkers measured an average depth of 9 mm in the superior-to-inferior direction of the glenoid.103 This depth is equivalent to approximately 40% of the radius of a typical 44-mm humeral replacement prosthesis. The anteroposterior depth of the glenoid measured an average of only 2.5 mm. However, these investigators thought that the anterior and posterior glenoid labrum added an additional 2.5 mm of depth. These data suggest that the labrum may be effective in increasing the depth of the glenoid and therefore has some contribution to articular stability. The humeral head needs to override the rim of the glenoid to dislocate. In other words, midrange stability depends on the depth of the glenoid to some extent. A cadaveric study revealed that removal of the entire anterior labrum without damaging the capsule resulted in increased translation of the humeral head in adduction (midrange instability) but did not alter the degree of stability in the anterior apprehension position (end-range stability).104
Fukuda and associates105 were the first to evaluate the relationship between the glenoid depth and stability in various kinds of shoulder prostheses. They used a ratio of a force necessary to dislocate the humeral component out of the glenoid socket to a force compressing the humeral component against the glenoid component to assess the inherent stability of the shoulder prosthesis. This ratio was constant in each type of prosthesis, and the deeper the glenoid socket, the greater the ratio. Later, Lippitt and associates106 termed this ratio the stability ratio. In normal shoulders, the stability ratio is 50% to 60% in the superior-to-inferior direction and 30% to 35% in the anterior-to-posterior direction. The stability ratio increases with an increase in glenoid depth. After the labrum is removed, the stability ratio decreases by approximately 20%. The stability ratio further decreases after creating a chondrolabral defect.37 According to Halder and colleagues,107 the stability ratio was greatest in the inferior direction (Fig. 6-29), and it was greater with the arm in adduction than in abduction. In shoulders with multidirectional instability, the stability ratio is decreased due to glenoid dysplasia. In surgical procedures, the stability ratio can be increased by 25% with use of capsulolabral augmentation78 and by 34% with use of glenoid osteotomy.108
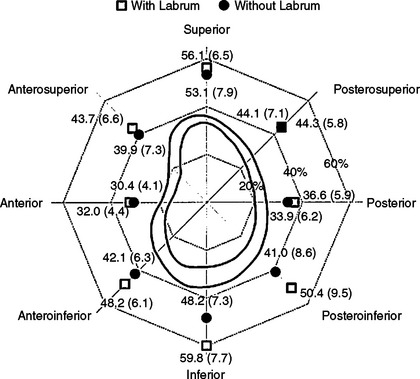
FIGURE 6-29 The average stability ratios of the shoulders, with and without the labrum, in eight tested directions. The ratios are defined as the peak translational force divided by the applied compressive force. The values are given as the average (and standard deviation).
(From Halder AM, Kuhl SG, Zobitz ME, et al: Effects of the glenoid labrum and glenohumeral abduction on stability of the shoulder joint through concavity-compression: An in vitro study. J Bone Joint Surg Am 7:1062-1069, 2001.)
Detachment of the superior aspect of the labrum from anterior and posterior is called a type II SLAP lesion. The pathogenesis of this lesion has been studied in the literature. Grauer and coworkers applied a 20-N force to the LHB and measured the strain in the anterior and posterior portions of the labrum.109 They found that strain was the greatest with the arm in full abduction and the smallest in adduction. Bey and colleagues applied a failure load to the LHB with the shoulder reduced and subluxated inferiorly.110 In reduced shoulders, the load created a type II SLAP lesion in two of eight shoulders, whereas in subluxated shoulders, the lesion developed in seven of eight shoulders.
Pradhan and associates simulated a throwing motion and measured strain on the anterosuperior and posterosuperior portions of the labrum in cadaveric shoulders.111 They found that the strain was greatest at the posterosuperior portion of the labrum when the arm was in abduction and external rotation (late cocking phase). Repetitive throwing motion can bring the superior labrum under constant strain and various degrees of shear force created by the cuff tendons during internal impingement, which can eventually result in detachment of the superior labrum from the glenoid. Once a type II SLAP lesion is created, a cadaveric study has shown that range of motion increases and the translation of the humeral head also increases.112,113
The joint contact area and position change during various glenohumeral motions are difficult to accurately measure by direct techniques. The contact point moves forward and inferior during internal rotation.14,48 With external rotation, the contact is just posteroinferior (Fig. 6-30). Saha reported that with elevation, the contact area moves superiorly. If elevation is combined with internal and external rotation, however, the humeral head remains centered in the glenoid as viewed in the axillary plane.53 The joint surface geometry and contact area have been measured with either stereophotogrammetry114 or electromagnetic tracking devices.115 In one study, the maximal contact area was obtained at 120 degrees of elevation. With an increase in arm elevation, the contact area shifted from an inferior region to a superocentral-posterior region, whereas the glenoid contact area shifted posteriorly (Fig. 6-31).114 Glenohumeral contact is maximal at functional positions (60-120 degrees of elevation) that provide stability to this joint.
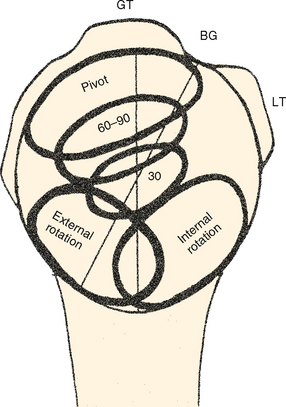
FIGURE 6-30 Humeral contact positions as a function of glenohumeral motion and positions. BG, bicipital groove; GT, greater tuberosity; LT, lesser tuberosity.
(Colorized from Nobuhara K: The Shoulder: Its Function and Clinical Aspects. Tokyo: Igaku-Shoin, 1987.)
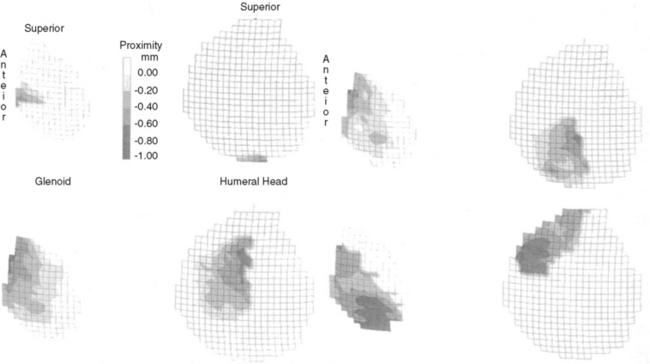
FIGURE 6-31 The contact area of the humeral head shifts from the inferior to the superocentral-posterior region with an increase in arm elevation, whereas the glenoid contact area shifts posteriorly.
(From Soslowsky LJ, Flatow EL, Bigliani LU, et al: Quantitation of in situ contact areas at the glenohumeral joint: A biomechanical study. J Orthop Res 10:524-534, 1992.)
Warner and colleagues measured the contact area by using Fuji prescale film. In adduction, the contact area of the humeral head on the glenoid was limited to the anatomic region of the central glenoid known as the bare area, whereas in abduction, the contact area as well as the congruity increased.116 They concluded that there was a slight articular mismatch in adduction but that it became more congruent and stable in abduction. Sahara and colleagues used open MRI to describe three-dimensional motion of the glenoid on the articular surface of the humeral head (Fig. 6-32).11 The glenoid was initially located at the inferior portion of the humeral head at 0 degrees of abduction. The glenoid shifted posteriorly from 0 to 60 degrees of abduction, moved up to the posterior-superior part of the humeral head from 60 to 120 degrees of abduction, and then moved anteriorly close to the bicipital groove at maximum abduction.
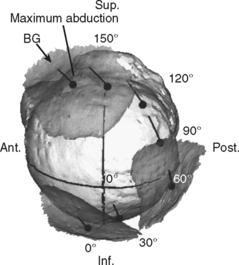
FIGURE 6-32 The glenoid movement relative to the humeral head. The semitransparent gray ellipse is the glenoid at 0 degrees, 60 degrees, and maximum abduction. The black dots are the centers of the glenoid at each abducted position. The bars indicate the superior directions of the long axis of the glenoid. Ant, anterior; BG, bicipital groove; Inf, inferior; Post, posterior; Sup, superior.
(From Sahara W, Sugamoto K, Murai M, Tanaka H: Yoshikawa H: The three-dimensional motions of glenohumeral joint under semi-loaded condition during arm abduction using vertically open MRI. Clin Biomech 22:304-312, 2007.)
The slight 5-degree superior tilt of the articular surface has been offered by Basmajian and Bazant as a factor in preventing inferior subluxation of the humerus when combined with the effect of the superior capsule and superior glenohumeral ligament (SGHL) (Fig. 6-33).47 Clinically, glenoid dysplasia with the glenoid facing downward is related to multidirectional instability of the shoulder.117 Glenoid osteotomy or pectoralis major transfer can be performed in shoulders with multidirectional instability to increase scapular inclination.55 A biomechanical study by Itoi and associates118 has clarified the relationship between scapular inclination and inferior stability of the shoulder. As the scapula was adducted (glenoid facing downward), all the vented shoulders dislocated inferiorly, whereas they were reduced with an increase in scapular abduction (Fig. 6-34). They further studied the bulk effect of the rotator cuff muscles on the stability provided by scapular inclination.119 After removal of the cuff muscles, the stability provided by scapular inclination did not change. Thus, the mechanism of scapular inclination seems to be a cam effect determined by the geometry of the glenoid and humerus and also by the length and orientation of the superior capsuloligamentous structures. Later, Metcalf and colleagues demonstrated that glenoid osteotomy and a 5-mm bone graft increased the stability ratio from 0.47 to 0.81 in the posteroinferior direction.108
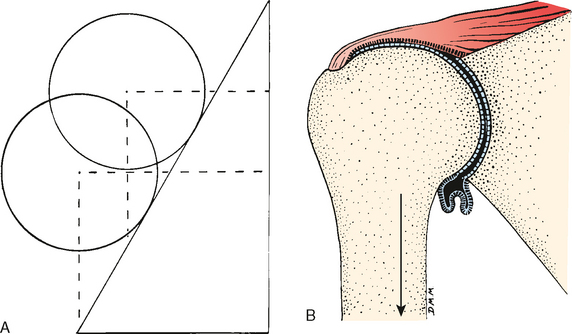
FIGURE 6-33 The upward tilt of the glenoid, coupled with the superior glenohumeral ligament and the coracohumeral ligament, resists passive downward displacement of the humeral head. A, Plane geometric diagram. B, Anatomic diagram.
(Modified from Basmajian JV, Bazant FJ: Factors preventing downward dislocation of the adducted shoulder joint. J Bone Joint Surg Am 41:1182-1186, 1959.)
Комментариев нет:
Отправить комментарий